Title
From Strain Selection to Purification - Key Factors for Successful Plasmid Production
Description
Plasmids serve as important tool in genetic engineering, gene therapy research or genetic vaccination. Recom- binant plasmid DNA (pDNA) is produced in bacterial cultures, mostly in *_Escherichia coli (E. coli)_. Successful pDNA production, reaching high yield and quality, relies on multiple factors, including the type of vector and host strain, th...
Products Used in Application
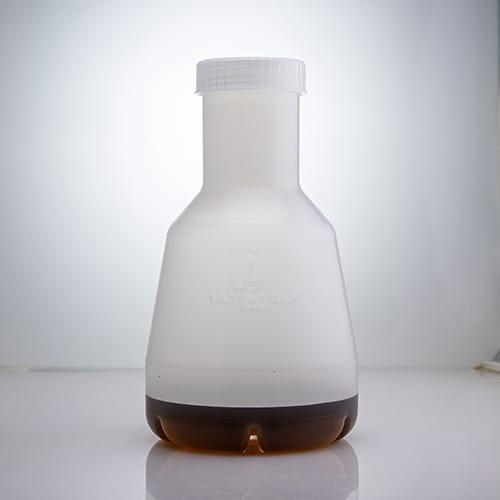
Ultra Yield® 2.5L Flask
500mL-1L Working Volume | Sterile
pn#931136-B
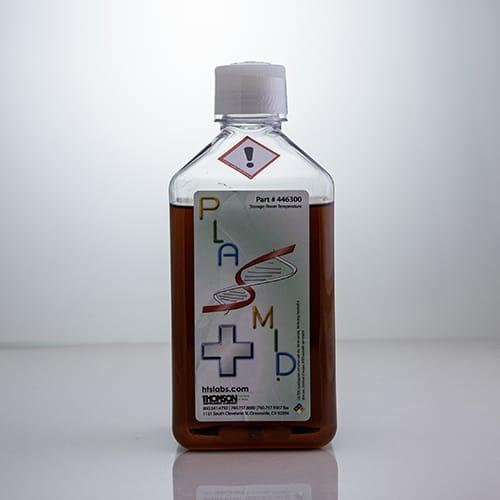
Plasmid+® Media
1L Bottle | Sterile
pn#446300
Related Products
From Strain Selection to Purification - Key Factors for Successful Plasmid Production
1Eppendorf SE, Jülich, Germany
2Eppendorf SE, Hamburg, Germany 3Promega, Madison, WI USA
4Thomson Instrument Company, Oceanside, CA USA
Plasmids serve as important tool in genetic engineering, gene therapy research or genetic vaccination. Recom- binant plasmid DNA (pDNA) is produced in bacterial cultures, mostly in *_Escherichia coli (E. coli)_. Successful pDNA production, reaching high yield and quality, relies on multiple factors, including the type of vector and host strain, the insert, and the methodology chosen for cultivation and downstream purification. Here, we review the distinct steps in the plasmid production workflow and give tips on optimization: from vector and strain selection to cultivation, from shake flasks to fermenters up to downstream processing steps of harvesting and purification.